Phosphorylation
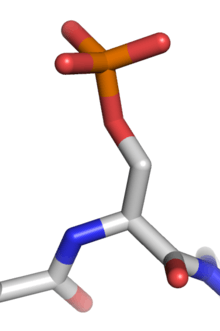
Phosphorylation is the addition of a phosphate group (PO4)3− to a molecule. In biology, phosphorylation and its counterpart, dephosphorylation, are critical for many cellular processes. A large fraction of proteins are at least temporarily phosphorylated, as are many sugars, lipids, and other molecules. Phosphorylation is especially important for protein function as this modification activates (or deactivates) many enzymes, thereby regulating their function. Protein phosphorylation is one type of post-translational modification.
The prominent role of protein phosphorylation in biochemistry is illustrated by the huge body of studies published on the subject (as of March 2015, the MEDLINE database returns over 240,000 articles, mostly on protein phosphorylation).
Phosphorylation of glucose
Phosphorylation of sugars is often the first stage of their catabolism. It allows cells to accumulate sugars because the phosphate group prevents the molecules from diffusing back across their transporter. Phosphorylation of glucose is a key reaction in sugar metabolism because many sugars are first converted to glucose before they are metabolized further.
The chemical equation for the conversion of D-glucose to D-glucose-6-phosphate in the first step of glycolysis is given by D-glucose + ATP -> D-glucose-6-phosphate + ATP ΔG° = -16.7 kJ.
Research D. G. Walker of the University of Birmingham determined the presence of two specific enzymes in adult guinea pig liver, both of which catalyze the phosphorylation of glucose to glucose 6 phosphate. The two enzymes have been identified as a specific glucokinase (ATP-D-glucose 6-phosphotransferase) and non-specific hexokinase (ATP-D-hexose 6-phosphotransferase).
Hepatic cell is freely permeable to glucose, and the initial rate of phosphorylation of glucose is the rate-limiting step in glucose metabolism by the liver (ATP-D-glucose 6-phosphotransferase) and non-specific hexokinase (ATP-D-hexose 6-phosphotransferase).[1]
The role of glucose 6-phosphate in glycogen synthase: High blood glucose concentration causes an increase in intracellular levels of glucose 6 phosphate in liver, skeletal muscle and fat (adipose) tissue. (ATP-D-glucose 6-phosphotransferase) and non-specific hexokinase (ATP-D-hexose 6-phosphotransferase). In liver, synthesis of glycogen is directly correlated by blood glucose concentration and in skeletal muscle and adipocytes, glucose has a minor effect on glycogen synthase. High blood glucose releases insulin, stimulating the trans location of specific glucose transporters to the cell membrane.[1][2]
The liver’s crucial role in controlling blood sugar concentrations by breaking down glucose into carbon dioxide and glycogen is characterized by the negative delta G value, which indicates that this is a point of regulation with. The hexokinase enzyme has a low Km, indicating a high affinity for glucose, so this initial phosphorylation can proceed even when glucose levels at nanoscopic scale within the blood.
The phosphorylation of glucose can be enhanced by the binding of Fructose-6-phosphate, and lessened by the binding fructose-1-phosphate. Fructose consumed in the diet is converted to F1P in the liver. This negates the action of F6P on glucokinase,[3] which ultimately favors the forward reaction. The capacity of liver cells to phosphorylate fructose exceeds capacity of metabolize fructose-1-phosphate. Consuming excess fructose ultimately results in an imbalance in liver metabolism, which indirectly exhausts the liver cell’s supply of ATP.[4]
Allosteric activation by glucose 6 phosphate, which acts as an effector, stimulates glycogen synthase, and glucose 6 phosphate may inhibit the phosphorylation of glycogen synthase by cyclic AMP-stimulated protein kinase.[2]
Phosphorylation of glucose is imperative in processes within the body. For example, phosphorylating glucose is necessary for insulin-dependent mechanistic target of rapamycin pathway activity within the heart. This further suggests a link between intermediary metabolism and cardiac growth.[5]
Protein phosphorylation
Function
Reversible phosphorylation of proteins is an important regulatory mechanism that occurs in both prokaryotic and eukaryotic organisms.[6][7][8][9] Kinases phosphorylate proteins and phosphatases dephosphorylate proteins. Many enzymes and receptors are switched "on" or "off" by phosphorylation and dephosphorylation. Reversible phosphorylation results in a conformational change in the structure in many enzymes and receptors, causing them to become activated or deactivated. Phosphorylation usually occurs on serine, threonine, tyrosine and histidine residues in eukaryotic proteins. Histidine phosphorylation of eukaryotic proteins appears to be much more frequent than tyrosine phosphorylation.[10] In prokaryotic proteins phosphorylation occurs on the serine, threonine, tyrosine, histidine or arginine or lysine residues.[6][7][10][11] The addition of a phosphate (PO4) molecule to a polar R group of an amino acid residue can turn a hydrophobic portion of a protein into a polar and extremely hydrophilic portion of molecule. In this way protein dynamics can induce a conformational change in the structure of the protein via long-range allostery with other hydrophobic and hydrophilic residues in the protein.
One such example of the regulatory role that phosphorylation plays is the p53 tumor suppressor protein. The p53 protein is heavily regulated[12] and contains more than 18 different phosphorylation sites. Activation of p53 can lead to cell cycle arrest, which can be reversed under some circumstances, or apoptotic cell death.[13] This activity occurs only in situations wherein the cell is damaged or physiology is disturbed in normal healthy individuals.
Upon the deactivating signal, the protein becomes dephosphorylated again and stops working. This is the mechanism in many forms of signal transduction, for example the way in which incoming light is processed in the light-sensitive cells of the retina.
Regulatory roles of phosphorylation include
- Biological thermodynamics of energy-requiring reactions
- Phosphorylation of Na+/K+-ATPase during the transport of sodium (Na+) and potassium (K+) ions across the cell membrane in osmoregulation to maintain homeostasis of the body's water content.
- Mediates enzyme inhibition
- Phosphorylation of the enzyme GSK-3 by AKT (Protein kinase B) as part of the insulin signaling pathway.[14]
- Phosphorylation of src tyrosine kinase (pronounced "sarc") by C-terminal Src kinase (Csk) induces a conformational change in the enzyme, resulting in a fold in the structure, which masks its kinase domain, and is thus shut "off".[15]
- Important for protein-protein interaction via "recognition domains."
- Phosphorylation of the cytosolic components of NADPH oxidase, a large membrane-bound, multi-protein enzyme present in phagocytic cells, plays an important role in the regulation of protein-protein interactions in the enzyme.[16]
- Important in protein degradation.
- In the late 1990s, it was recognized that phosphorylation of some proteins causes them to be degraded by the ATP-dependent ubiquitin/proteasome pathway. These target proteins become substrates for particular E3 ubiquitin ligases only when they are phosphorylated.
- Important in glycolysis.
Glycolysis is an essential process of glucose degrading into two molecules of pyruvate, through various steps, with the help of different enzymes. It occurs in ten steps and proves that phosphorylation is a much required and necessary to attain the end products. Phosphorylation initiates the reaction in step 1 of the preparatory step[17] (first half of glycolysis), and initiates step 6 of payoff phase (second phase of glycolysis).[18]
Glucose, by nature, is a small molecule with the ability to diffuse in and out of the cell. By phosphorylating glucose (adding a negatively charged phosphate group), glucose is converted to glucose-6-phosphate and trapped within the cell as the cell membrane is negatively charged. This reaction occurs due to the enzyme hexokinase, an enzyme that helps phosphorylate many six-membered ring structures. Glucose-6-phosphate cannot travel through the cell membrane and is therefore, coerced to stay inside the cell. Phosphorylation takes place in step 3, where fructose-6-phosphate is converted to fructose-1,6-bisphosphate. This reaction is catalyzed by phosphofructokinase.
While phosphorylation is performed by ATPs during preparatory steps, phosphorylation during payoff phase is maintained by inorganic phosphate. Each molecule of glyceraldehyde-3-phosphate is phosphorylated to form 1,3-bisphosphoglycerate. This reaction is catalyzed by GAPDH (glyceraldehyde-3-phosphate dehydrogenase). The cascade effect of phosphorylation eventually causes instability and allows enzymes to open the carbon bonds in glucose.
Phosphorylation functions as an extremely vital component of glycolysis, for it helps in transport, control and efficiency.[19]
Signaling networks
Elucidating complex signaling pathway phosphorylation events can be difficult. In cellular signaling pathways, protein A phosphorylates protein B, and B phosphorylates C. However, in another signaling pathway, protein D phosphorylates A, or phosphorylates protein C. Global approaches such as phosphoproteomics, the study of phosphorylated proteins, which is a sub-branch of proteomics, combined with mass spectrometry-based proteomics, have been utilised to identify and quantify dynamic changes in phosphorylated proteins over time. These techniques are becoming increasingly important for the systematic analysis of complex phosphorylation networks.[20] They have been successfully used to identify dynamic changes in the phosphorylation status of more than 6000 sites after stimulation with epidermal growth factor.[21] Another approach for understanding Phosphorylation Network, is by measuring the genetic interactions between multiple phosphorylating proteins and their targets. This reveals interesting recurring patterns of interactions – network motifs.[22] Computational methods have been developed to model phosphorylation networks [23][24] and predict their responses under different perturbations.[25]
Types of phosphorylation
See also kinases for more details on the different types of phosphorylation
Within a protein, phosphorylation can occur on several amino acids. Phosphorylation on serine is the most common, followed by threonine. Tyrosine phosphorylation is relatively rare but is at the origin of protein phosphorylation signaling pathways in most of the eukaryotes. Phosphorylation on amino acids, such as serine, threonine, and tyrosine results in the formation of a phosphoprotein, when the phosphate group of the phosphoprotein reacts with the -OH group of a Ser, Thr, or Tyr sidechain in an esterification reaction.[26] However, since tyrosine phosphorylated proteins are relatively easy to purify using antibodies, tyrosine phosphorylation sites are relatively well understood. Histidine and aspartate phosphorylation occurs in prokaryotes as part of two-component signaling and in some cases in eukaryotes in some signal transduction pathways.[27] In prokaryotes, archea, and some lower eukaryotes histidine's nitrogen act as a nucleophile and binds to a phosphate group.[28] Once histidine is phosphorylated the regulatory domain of the response regulator catalyzes the transfer of the phosphate to aspartate.
Phosphorylation of histones
Eukaryotic DNA is organized with histone proteins in specific complexes called chromatin. The chromatin structure functions and facilitates the packaging, organization and distribution of eukaryotic DNA. However, it has a negative impact on several fundamental biological processes such as transcription, replication and DNA repair by restricting the accessibility of certain enzymes and proteins. Post-translational modification of histones such as histone phosphorylation has been shown to modify the chromatin structure by changing protein:DNA or protein:protein interactions.[29] Histone post-translational modifications modify the chromatin structure. The most commonly associated histone phosphorylation occurs during cellular responses to DNA damage, when phosphorylated histone H2A separates large chromatin domains around the site of DNA breakage.[30] Researchers investigated whether modifications of histones directly impact RNA polymerase II directed transcription. Researchers choose proteins that are known to modify histones to test their effects on transcription, and found that the stress-induced kinase, MSK1, inhibits RNA synthesis. Inhibition of transcription by MSK1 was most sensitive when the template was in chromatin, since DNA templates not in chromatin were resistant to the effects of MSK1. It was shown that MSK1 phosphorylated histone H2A on serine 1, and mutation of serine 1 to alanine blocked the inhibition of transcription by MSK1. Thus results suggested that the acetylation of histones can stimulate transcription by suppressing an inhibitory phosphorylation by a kinase as MSK1.[31]
Detection and characterization
Antibodies can be used as powerful tool to detect whether a protein is phosphorylated at a particular site. Antibodies bind to and detect phosphorylation-induced conformational changes in the protein. Such antibodies are called phospho-specific antibodies; hundreds of such antibodies are now available. They are becoming critical reagents both for basic research and for clinical diagnosis.

Posttranslational modification (PTM) isoforms are easily detected on 2D gels. Indeed, phosphorylation replaces neutral hydroxyl groups on serines, threonines, or tyrosines with negatively charged phosphates with pKs near 1.2 and 6.5. Thus, below pH 5.5, phosphates add a single negative charge; near pH 6.5, they add 1.5 negative charges; above pH 7.5, they add 2 negative charges. The relative amount of each isoform can also easily and rapidly be determined from staining intensity on 2D gels.
In some very specific cases, the detection of the phosphorylation as a shift in the protein's electrophoretic mobility is possible on simple 1-dimensional SDS-PAGE gels, as it's described for instance for a transcriptional coactivator by Kovacs et al.[32] Strong phosphorylation-related conformational changes (that persist in detergent-containing solutions) are thought to underlie this phenomenon. Most of the phosphorylation sites for which such a mobility shift has been described fall in the category of SP and TP sites (i.e. a proline residue follows the phosphorylated serine or threonine residue).
More recently large-scale mass spectrometry analyses have been used to determine sites of protein phosphorylation. Over the last 4 years, dozens of studies have been published, each identifying thousands of sites, many of which were previously undescribed.[33][34] Mass spectrometry is ideally suited for such analyses using HCD or ETD fragmentation, as the addition of phosphorylation results in an increase in the mass of the protein and the phosphorylated residue. Advanced, highly accurate mass spectrometers are needed for these studies, limiting the technology to labs with high-end mass spectrometers. However, the analysis of phosphorylated peptides by mass spectrometry is still not as straightforward as for “regular”, unmodified peptides. Recently EThcD has been developed combining electron-transfer and higher-energy collision dissociation. Compared to the usual fragmentation methods, EThcD scheme provides more informative MS/MS spectra for unambiguous phosphosite localization.[35]
A detailed characterization of the sites of phosphorylation is very difficult, and the quantitation of protein phosphorylation by mass spectrometry requires isotopic internal standard approaches.[36] A relative quantitation can be obtained with a variety of differential isotope labeling technologies.[37] There are also several quantitative protein phosphorylation methods, including fluorescence immunoassays, Microscale thermophoresis, FRET, TRF, fluorescence polarization, fluorescence-quenching, mobility shift, bead-based detection, and cell-based formats.[38][39]
ATP
ATP, the "high-energy" exchange medium in the cell, is synthesized in the mitochondrion by addition of a third phosphate group to ADP in a process referred to as oxidative phosphorylation. ATP is also synthesized by substrate-level phosphorylation during glycolysis. ATP is synthesized at the expense of solar energy by photophosphorylation in the chloroplasts of plant cells.
See also
References
- 1 2 Walker DG, Rao S (1964). "The role of glucokinase in the phosphorylation of glucose by rat liver". Biochemical Journal. 90 (2): 361.
- 1 2 Villar-Palasí, C.; Guinovart, J. J. (1 June 1997). "The role of glucose 6-phosphate in the control of glycogen synthase.". The FASEB Journal. 11 (7): 544–558. ISSN 0892-6638.
- ↑ Walker DG, Rao S (1964). "The role of glucokinase in the phosphorylation of glucose by rat liver". Biochemical Journal. 90 (2): 360–368. doi:10.1042/bj0900360.
- ↑ "Regulation of Glycolysis:". cmgm.stanford.edu. Retrieved 2016-05-19.
- ↑ Sharma, Saumya; Guthrie, Patrick H.; Chan, Suzanne S.; Haq, Syed; Taegtmeyer, Heinrich (2007-10-01). "Glucose phosphorylation is required for insulin-dependent mTOR signalling in the heart". Cardiovascular Research. 76 (1): 71–80. doi:10.1016/j.cardiores.2007.05.004. ISSN 0008-6363. PMC 2257479
. PMID 17553476.
- 1 2 Cozzone AJ (1988). "Protein phosphorylation in prokaryotes". Annu. Rev. Microbiol. 42: 97–125. doi:10.1146/annurev.mi.42.100188.000525. PMID 2849375.
- 1 2 Stock JB, Ninfa AJ, Stock AM (December 1989). "Protein phosphorylation and regulation of adaptive responses in bacteria". Microbiol. Rev. 53 (4): 450–90. PMC 372749
. PMID 2556636.
- ↑ Chang C, Stewart RC (July 1998). "The Two-Component System . Regulation of Diverse Signaling Pathways in Prokaryotes and Eukaryotes". Plant Physiol. 117 (3): 723–31. doi:10.1104/pp.117.3.723. PMC 1539182
. PMID 9662515.
- ↑ Barford D, Das AK, Egloff MP (1998). "The structure and mechanism of protein phosphatases: insights into catalysis and regulation". Annu Rev Biophys Biomol Struct. 27: 133–64. doi:10.1146/annurev.biophys.27.1.133. PMID 9646865.
- 1 2 Ciesla J, Fraczyk T, Rode W (2011). "Phosphorylation of basic amino acid residues in proteins: important but easily missed". Acta Biochim Pol. 58 (2): 137–47. PMID 21623415.
- ↑ Deutscher, J.; Saier, J. (2005). "Ser/Thr/Tyr Protein Phosphorylation in Bacteria – for Long Time Neglected, Now Well Established". Journal of Molecular Microbiology and Biotechnology. 9 (3–4): 125–131. doi:10.1159/000089641. PMID 16415586.
- ↑ Ashcroft M, Kubbutat MH, Vousden KH (March 1999). "Regulation of p53 Function and Stability by Phosphorylation". Mol. Cell. Biol. 19 (3): 1751–8. doi:10.1128/mcb.19.3.1751. PMC 83968
. PMID 10022862.
- ↑ Bates S, Vousden KH (February 1996). "p53 in signaling checkpoint arrest or apoptosis". Curr. Opin. Genet. Dev. 6 (1): 12–8. doi:10.1016/S0959-437X(96)90004-0. PMID 8791489.
- ↑ van Weeren PC, de Bruyn KM, de Vries-Smits AM, van Lint J, Burgering BM (May 1998). "Essential role for protein kinase B (PKB) in insulin-induced glycogen synthase kinase 3 inactivation. Characterization of dominant-negative mutant of PKB". J. Biol. Chem. 273 (21): 13150–6. doi:10.1074/jbc.273.21.13150. PMID 9582355.
- ↑ Cole PA, Shen K, Qiao Y, Wang D (October 2003). "Protein tyrosine kinases Src and Csk: a tail's tale". Curr Opin Chem Biol. 7 (5): 580–5. doi:10.1016/j.cbpa.2003.08.009. PMID 14580561.
- ↑ Babior BM (March 1999). "NADPH oxidase: an update". Blood. 93 (5): 1464–76. PMID 10029572.
- ↑ "Chapter 14: Glycolysis and the Catabolism of Hexoses".
- ↑ Garrett, Reginald (1995). Biochemistry. Saunders College.
- ↑ "Introduction to Glycolysis".
- ↑ Olsen JV, Blagoev B, Gnad F, Macek B, Kumar C, Mortensen P, Mann M (November 2006). "Global, in vivo, and site-specific phosphorylation dynamics in signaling networks". Cell. 127 (3): 635–48. doi:10.1016/j.cell.2006.09.026. PMID 17081983.
- ↑ Li-Rong Y, Issaq HJ, Veenstra TD (2007). "Phosphoproteomics for the discovery of kinases as cancer biomarkers and drug targets". Proteomics: Clinical Applications. 1 (9): 1042–1057. doi:10.1002/prca.200700102. PMID 21136756.
- ↑ Fiedler D, Braberg H, Mehta M, Chechik G, Cagney G, Mukherjee P, Silva AC, Shales M, et al. (2009). "Functional Organization of the S. cerevisiae Phosphorylation Network". Cell. 136 (5): 952–963. doi:10.1016/j.cell.2008.12.039. PMC 2856666
. PMID 19269370.
- ↑ Schoeberl, B; Eichler-Jonsson, C; Gilles, ED; Müller, G (Apr 2002). "Computational modeling of the dynamics of the MAP kinase cascade activated by surface and internalized EGF receptors.". Nature Biotechnology. 20 (4): 370–5. doi:10.1038/nbt0402-370. PMID 11923843.
- ↑ Aldridge, BB; Burke, JM; Lauffenburger, DA; Sorger, PK (Nov 2006). "Physicochemical modelling of cell signalling pathways.". Nature Cell Biology. 8 (11): 1195–203. doi:10.1038/ncb1497. PMID 17060902.
- ↑ Zhu, F; Guan, Y (Jun 11, 2014). "Predicting Dynamic Signaling Network Response under Unseen Perturbations". Bioinformatics (Oxford, England). 30 (19): 2772–8. doi:10.1093/bioinformatics/btu382. PMC 4173019
. PMID 24919880.
- ↑ Grisham, Reginald H. Garrett, Charles M. (2013). Biochemistry (5th ed.). Belmont, CA: Brooks/Cole, Cengage Learning. ISBN 978-1133106296.
- ↑ Thomason P, Kay R (September 2000). "Eukaryotic signal transduction via histidine-aspartate phosphorelay" (PDF). J. Cell. Sci. 113 (18): 3141–50. PMID 10954413.
- ↑ Puttick, Jennifer; Baker, Edward N.; Delbaere, Louis T.J. (2008). "Histidine phosphorylation in biological systems". Biochimica et Biophysica Acta (BBA) - Proteins and Proteomics. 1784 (1): 100–105. doi:10.1016/j.bbapap.2007.07.008. ISSN 1570-9639.
- ↑ Sawicka, Anna; Seiser, Christian (2014-08-01). "Sensing core histone phosphorylation — A matter of perfect timing". Biochimica et Biophysica Acta (BBA) - Gene Regulatory Mechanisms. Molecular mechanisms of histone modification function. 1839 (8): 711–718. doi:10.1016/j.bbagrm.2014.04.013. PMC 4103482
. PMID 24747175.
- ↑ Rossetto, Dorine; Avvakumov, Nikita; Côté, Jacques (2012-10-01). "Histone phosphorylation". Epigenetics. 7 (10): 1098–1108. doi:10.4161/epi.21975. ISSN 1559-2294. PMC 3469451
. PMID 22948226.
- ↑ Zhang, Ye; Griffin, Karen; Mondal, Neelima; Parvin, Jeffrey D. (2004-05-21). "Phosphorylation of Histone H2A Inhibits Transcription on Chromatin Templates". Journal of Biological Chemistry. 279 (21): 21866–21872. doi:10.1074/jbc.M400099200. ISSN 0021-9258. PMID 15010469.
- ↑ Kovacs KA, Steinmann M; Magistretti PJ; Halfon O; Cardinaux JR (Sep 2003). "CCAAT/enhancer-binding protein family members recruit the coactivator CREB-binding protein and trigger its phosphorylation". J. Biol. Chem. UNITED STATES. 278 (38): 36959–65. doi:10.1074/jbc.M303147200. ISSN 0021-9258. PMID 12857754.
- ↑ Munton RP, Tweedie-Cullen R, Livingstone-Zatchej M, Weinandy F, Waidelich M, Longo D, Gehrig P, Potthast F, et al. (February 2007). "Qualitative and quantitative analyses of protein phosphorylation in naive and stimulated mouse synaptosomal preparations". Mol. Cell Proteomics. 6 (2): 283–93. doi:10.1074/mcp.M600046-MCP200. PMID 17114649.
- ↑ Trinidad JC, Thalhammer A, Specht CG, Lynn AJ, Baker PR, Schoepfer R, Burlingame AL (April 2008). "Quantitative analysis of synaptic phosphorylation and protein expression". Mol. Cell Proteomics. 7 (4): 684–96. doi:10.1074/mcp.M700170-MCP200. PMID 18056256.
- ↑ Frese, Christian; Houjiang Zhou; Thomas Taus; A. F. Maarten Altelaar; Karl Mechtler; Albert J. R. Heck; Shabaz Mohammed (March 1, 2013). "Unambiguous Phosphosite Localization using Electron-Transfer/Higher-Energy Collision Dissociation (EThcD)". J Proteome Res. 12 (3): 1520–1525. doi:10.1021/pr301130k. PMC 3588588
. PMID 23347405.
- ↑ Gerber SA, Rush J, Stemman O, Kirschner MW, Gygi SP (June 2003). "Absolute quantification of proteins and phosphoproteins from cell lysates by tandem MS". Proc. Natl. Acad. Sci. U.S.A. 100 (12): 6940–5. Bibcode:2003PNAS..100.6940G. doi:10.1073/pnas.0832254100. PMC 165809
. PMID 12771378.
- ↑ Gygi SP, Rist B, Griffin TJ, Eng J, Aebersold R (2002). "Proteome analysis of low-abundance proteins using multidimensional chromatography and isotope-coded affinity tags". J. Proteome Res. 1 (1): 47–54. doi:10.1021/pr015509n. PMID 12643526.
- ↑ Olive DM (October 2004). "Quantitative methods for the analysis of protein phosphorylation in drug development". Expert Rev Proteomics. 1 (3): 327–41. doi:10.1586/14789450.1.3.327. PMID 15966829.
- ↑ Chen H, Kovar J, Sissons S, Cox K, Matter W, Chadwell F, Luan P, Vlahos CJ, et al. (March 2005). "A cell-based immunocytockemical assay for monitoring kinase signaling pathways and drug efficacy". Anal. Biochem. 338 (1): 136–42. doi:10.1016/j.ab.2004.11.015. PMID 15707944.